纳米材料的非毒性环境效应研究进展
Research Progress on Non-toxic Environmental Effects of Nanomaterials
-
摘要: 纳米材料的广泛应用使其不可避免地释放到环境中,并对生态环境产生潜在不利影响。但是纳米材料的实际环境浓度较低,是否会对生态安全造成严重毒性危害依然存在争议。因此,近年来纳米材料对生物体无明显生长抑制或毒性胁迫表型情况下的非毒性环境效应逐渐引起人们的关注。笔者综述了近年来纳米材料非毒性环境效应研究的最新进展,探讨了纳米材料对植物及微生物的非毒性胁迫影响机制,旨在加深人们对纳米材料实际环境效应的理解,并为相关领域的科学研究提供新的思路。Abstract: The wide application of nanomaterials has led to their inevitable release into the environment, causing adverse ecological impacts to environment. However, the actual concentrations of nanomaterials in environment are relatively low, and whether they cause serious toxic stress to ecological safety remains controversial. Therefore, the non-toxic environmental effects of nanomaterials on organisms without significant growth inhibition or toxic stress phenotypes have attracted increasing attention in recent years. This review summarizes the recent advance in the research on the non-toxic environmental effects of nanomaterials and discusses the mechanism of non-stress effects of nanomaterials on plants and microorganisms, aiming to have a better understanding of the actual environmental effects of nanomaterials and provide new insights for scientific research in related fields.
-
Key words:
- nanomaterials /
- plant /
- microorganism /
- non-toxic effects
-
Wang Y, Chou S, Zhang Z. Nanomaterials innovation[J]. Small, 2019, 15(32):1902246 Rezvani E, Rafferty A, McGuinness C, et al. Adverse effects of nanosilver on human health and the environment[J]. Acta Biomaterialia, 2019, 94:145-159 Sun T Y,Bornhöft N A, Hungerbühler K, et al. Dynamic probabilistic modeling of environmental emissions of engineered nanomaterials[J]. Environmental Science & Technology, 2016, 50(9):4701-4711 Leareng S K, Ubomba-Jaswa E, Musee N. Toxicity of zinc oxide and iron oxide engineered nanoparticles to Bacillus subtilis in river water systems[J]. Environmental Science:Nano, 2020, 7(1):172-185 Zhao J, Lin M Q, Wang Z Y, et al. Engineered nanomaterials in the environment:Are they safe?[J]. Critical Reviews in Environmental Science and Technology, 2020:1-36. doi:10.1080/10643389.2020.1764279 Ganguly P, Breen A, Pillai S C. Toxicity of nanomaterials:Exposure, pathways, assessment, and recent advances[J]. ACS Biomaterials Science & Engineering, 2018, 4(7):2237-2275 韩雪, 马晓琳, 晁韶良, 等. 纳米材料对环境抗生素抗性基因污染扩散影响的研究进展[J]. 生态毒理学报, 2019, 14(5):46-54 Han X, Ma X L, Chao S L, et al. Influence of nanomaterials on the spread of environmental antibiotic resistance genes:A review[J]. Asian Journal of Ecotoxicology, 2019, 14(5):46-54(in Chinese)
Wang D L, Lin Z F, Wang T, et al. Where does the toxicity of metal oxide nanoparticles come from:The nanoparticles, the ions, or a combination of both?[J]. Journal of Hazardous Materials, 2016, 308:328-334 Gottschalk F, Sun T Y, Nowack B. Environmental concentrations of engineered nanomaterials:Review of modeling and analytical studies[J]. Environmental Pollution, 2013, 181:287-300 Pérez-De-luque A. Interaction of nanomaterials with plants:What do we need for real applications in agriculture?[J]. Frontiers in Environmental Science, 2017, 5:12 Rifna E J, Ratish Ramanan K, Mahendran R. Emerging technology applications for improving seed germination[J]. Trends in Food Science & Technology, 2019, 86:95-108 Bandyopadhyay S, Plascencia-Villa G, Mukherjee A, et al. Comparative phytotoxicity of ZnO NPs, bulk ZnO, and ionic zinc onto the alfalfa plants symbiotically associated with Sinorhizobium meliloti in soil[J]. Science of the Total Environment, 2015, 515-516:60-69 Acharya P, Jayaprakasha G K, Crosby K M, et al. Nanoparticle-mediated seed priming improves germination, growth, yield, and quality of watermelons (Citrullus lanatus) at multi-locations in texas[J]. Scientific Reports, 2020, 10(1):5037 Zhang M, Gao B, Chen J J, et al. Effects of graphene on seed germination and seedling growth[J]. Journal of Nanoparticle Research, 2015, 17(2):78 Kumar V, Guleria P, Kumar V, et al. Gold nanoparticle exposure induces growth and yield enhancement in Arabidopsis thaliana[J]. Science of the Total Environment, 2013, 461-462:462-468 文双喜, 王毅力. 水培实验中不同粒径纳米TiO2对芦苇种子发芽和植株生长和生理的影响[J]. 生态毒理学报, 2017, 12(2):71-80 Wen S X, Wang Y L. Effect of nano titanium dioxide with different particle size on the seed germination and plant growth and physiology of Phragmites australis in hydroponic experiments[J]. Asian Journal of Ecotoxicology, 2017, 12(2):71-80(in Chinese)
Pandey K, Lahiani M H, Hicks V K, et al. Effects of carbon-based nanomaterials on seed germination, biomass accumulation and salt stress response of bioenergy crops[J]. PLoS One, 2018, 13(8):e0202274 Khodakovskaya M, Dervishi E, Mahmood M, et al. Carbon nanotubes are able to penetrate plant seed coat and dramatically affect seed germination and plant growth[J]. ACS Nano, 2009, 3(10):3221-3227 Oleszczuk P, Czech B, Kończak M, et al. Impact of ZnO and ZnS nanoparticles in sewage sludge-amended soil on bacteria, plant and invertebrates[J]. Chemosphere, 2019, 237:124359 Liu R Q, Zhang H Y, Lal R. Effects of stabilized nanoparticles of copper, zinc, manganese, and iron oxides in low concentrations on lettuce (Lactuca sativa) seed germination:Nanotoxicants or nanonutrients?[J]. Water, Air, & Soil Pollution, 2016, 227(1):1-14 Seddighinia F S, Iranbakhsh A, Oraghi Ardebili Z, et al. Seed priming with cold plasma and multi-walled carbon nanotubes modified growth, tissue differentiation, anatomy, and yield in bitter melon (Momordica charantia)[J]. Journal of Plant Growth Regulation, 2020, 39(1):87-98 Wang Y Y, Zhang P, Li M S, et al. Alleviation of nitrogen stress in rice (Oryza sativa) by ceria nanoparticles[J]. Environmental Science:Nano, 2020, 7(10):2930-2940 Ye Y, Cota-Ruiz K, Hernández-Viezcas J A, et al. Manganese nanoparticles control salinity-modulated molecular responses in Capsicum annuum L. through priming:A sustainable approach for agriculture[J]. ACS Sustainable Chemistry & Engineering, 2020, 8(3):1427-1436 Kim J H, Oh Y, Yoon H, et al. Iron nanoparticle-induced activation of plasma membrane H(+)-ATPase promotes stomatal opening in Arabidopsis thaliana[J]. Environmental Science & Technology, 2015, 49(2):1113-1119 Rizwan M, Ali S, Ali B, et al. Zinc and iron oxide nanoparticles improved the plant growth and reduced the oxidative stress and cadmium concentration in wheat[J]. Chemosphere, 2019, 214:269-277 Dai Y, Chen F, Yue L, et al. Uptake, transport, and transformation of CeO2 nanoparticles by strawberry and their impact on the rhizosphere bacterial community[J]. ACS Sustainable Chemistry & Engineering, 2020, 8(12):4792-4800 Wan J P, Wang R L, Bai H R, et al. Comparative physiological and metabolomics analysis reveals that single-walled carbon nanohorns and ZnO nanoparticles affect salt tolerance in Sophora alopecuroides[J]. Environmental Science:Nano, 2020, 7(10):2968-2981 Zhao D Q, Fang Z W, Tang Y H, et al. Graphene oxide as an effective soil water retention agent can confer drought stress tolerance to Paeonia ostii without toxicity[J]. Environmental Science & Technology, 2020, 54(13):8269-8279 Rizwan M, Ali S, Zia Ur Rehman M, et al. Alleviation of cadmium accumulation in maize (Zea mays L.) by foliar spray of zinc oxide nanoparticles and biochar to contaminated soil[J]. Environmental Pollution, 2019, 248:358-367 孙耀琴, 申聪聪, 葛源. 典型纳米材料的土壤微生物效应研究进展[J]. 生态毒理学报, 2016, 11(5):2-13 Sun Y Q, Shen C C, Ge Y. Review on microbiological effects of typical nanomaterials in soil ecosystem[J]. Asian Journal of Ecotoxicology, 2016, 11(5):2-13(in Chinese)
Guan X Y, Gao X Y, Avellan A, et al. CuO nanoparticles alter the rhizospheric bacterial community and local nitrogen cycling for wheat grown in a calcareous soil[J]. Environmental Science & Technology, 2020, 54(14):8699-8709 Shang H P, Ma C X, Li C Y, et al. Copper sulfide nanoparticles suppress Gibberella fujikuroi infection in rice (Oryza sativa L.) by multiple mechanisms:Contact-mortality, nutritional modulation and phytohormone regulation[J]. Environmental Science:Nano, 2020, 7(9):2632-2643 Xu J B, Luo X S, Wang Y L, et al. Evaluation of zinc oxide nanoparticles on lettuce (Lactuca sativa L.) growth and soil bacterial community[J]. Environmental Science and Pollution Research International, 2018, 25(6):6026-6035 Rico C M, Barrios A C, Tan W J, et al. Physiological and biochemical response of soil-grown barley (Hordeum vulgare L.) to cerium oxide nanoparticles[J]. Environmental Science and Pollution Research International, 2015, 22(14):10551-10558 Wu H H, Shabala L, Shabala S, et al. Hydroxyl radical scavenging by cerium oxide nanoparticles improves Arabidopsis salinity tolerance by enhancing leaf mesophyll potassium retention[J]. Environmental Science:Nano, 2018, 5(7):1567-1583 Rui M M, Ma C X, Hao Y, et al. Iron oxide nanoparticles as a potential iron fertilizer for peanut (Arachis hypogaea)[J]. Frontiers in Plant Science, 2016, 7:815 Adhikari T, Sarkar D, Mashayekhi H, et al. Growth and enzymatic activity of maize (Zea mays L.) plant:Solution culture test for copper dioxide nano particles[J]. Journal of Plant Nutrition, 2016, 39(1):99-115 Tian L Y, Zhang H L, Zhao X P, et al. CdS nanoparticles in soil induce metabolic reprogramming in broad bean (Vicia faba L.) roots and leaves[J]. Environmental Science:Nano, 2020, 7(1):93-104 Abdulla Abdulaziz Alshehddi L, Bokhari N. Influence of gold and silver nanoparticles on the germination and growth of Mimusops laurifolia seeds in the South-Western regions in Saudi Arabia[J]. Saudi Journal of Biological Sciences, 2020, 27(1):574-580 Wang L K, Sun J Z, Lin L M, et al. Silver nanoparticles regulate Arabidopsis root growth by concentration-dependent modification of reactive oxygen species accumulation and cell division[J]. Ecotoxicology and Environmental Safety, 2020, 190:110072 Hernández H H, Benavides-Mendoza A, Ortega-Ortiz H, et al. Cu nanoparticles in chitosan-PVA hydrogels as promoters of growth, productivity and fruit quality in tomato[J]. Emirates Journal of Food and Agriculture, 2017, 29(8):573-580 Rahmani N, Radjabian T, Soltani B M. Impacts of foliar exposure to multi-walled carbon nanotubes on physiological and molecular traits of Salvia verticillata L., as a medicinal plant[J]. Plant Physiology and Biochemistry, 2020, 150:27-38 Qiu Z G, Yu Y M, Chen Z L, et al. Nanoalumina promotes the horizontal transfer of multiresistance genes mediated by plasmids across genera[J]. Proceedings of the National Academy of Sciences of the United States of America, 2012, 109(13):4944-4949 Liu X M, Tang J C, Song B R, et al. Exposure to Al2O3 nanoparticles facilitates conjugative transfer of antibiotic resistance genes from Escherichia coli to Streptomyces[J]. Nanotoxicology, 2019, 13(10):1422-1436 Lu J, Wang Y, Jin M, et al. Both silver ions and silver nanoparticles facilitate the horizontal transfer of plasmid-mediated antibiotic resistance genes[J]. Water Research, 2020, 169:115229 Han X, Lv P, Wang L G, et al. Impact of nano-TiO2 on horizontal transfer of resistance genes mediated by filamentous phage transduction[J]. Environmental Science:Nano, 2020, 7(4):1214-1224 Li G Y, Chen X F, Yin H L, et al. Natural sphalerite nanoparticles can accelerate horizontal transfer of plasmid-mediated antibiotic-resistance genes[J]. Environment International, 2020, 136:105497 Zhang Y R, Yang Z H, Xiang Y P, et al. Evolutions of antibiotic resistance genes (ARGs), class 1integron-integrase (intⅠ1) and potential hosts of ARGs during sludge anaerobic digestion with the iron nanoparticles addition[J]. Science of the Total Environment, 2020, 724:138248 Xiang Y P, Yang Z H, Zhang Y R, et al. Influence of nanoscale zero-valent iron and magnetite nanoparticles on anaerobic digestion performance and macrolide, aminoglycoside, β-lactam resistance genes reduction[J]. Bioresource Technology, 2019, 294:122139 Chen Y R, Guo X P, Feng J N, et al. Impact of ZnO nanoparticles on the antibiotic resistance genes (ARGs) in estuarine water:ARG variations and their association with the microbial community[J]. Environmental Science:Nano, 2019, 6(8):2405-2419 Hu X J, Yang B, Zhang W, et al. Plasmid binding to metal oxide nanoparticles inhibited lateral transfer of antibiotic resistance genes[J]. Environmental Science:Nano, 2019, 6(5):1310-1322 Ding C S, Pan J, Jin M, et al. Enhanced uptake of antibiotic resistance genes in the presence of nanoalumina[J]. Nanotoxicology, 2016, 10(8):1051-1060 Zhang Y, Gu A Z, Cen T, et al. Sub-inhibitory concentrations of heavy metals facilitate the horizontal transfer of plasmid-mediated antibiotic resistance genes in water environment[J]. Environmental Pollution, 2018, 237:74-82 Huang H N, Chen Y G, Yang S Y, et al. CuO and ZnO nanoparticles drive the propagation of antibiotic resistance genes during sludge anaerobic digestion:Possible role of stimulated signal transduction[J]. Environmental Science:Nano, 2019, 6(2):528-539 陆贤, 郭美婷, 张伟贤. 纳米零价铁对耐四环素菌耐药特性的影响[J]. 中国环境科学, 2017, 37(1):381-385 Lu X, Guo M T, Zhang W X. Influence of nanoscale zero-valent iron (nZVI) on resistance character of tetracycline resistant bacteria[J]. China Environmental Science, 2017, 37(1):381-385(in Chinese)
Zhang S, Wang Y, Song H L, et al. Copper nanoparticles and copper ions promote horizontal transfer of plasmid-mediated multi-antibiotic resistance genes across bacterial genera[J]. Environment International, 2019, 129:478-487 Wang X L, Yang F X, Zhao J, et al. Bacterial exposure to ZnO nanoparticles facilitates horizontal transfer of antibiotic resistance genes[J]. NanoImpact, 2018, 10:61-67 Guo M T, Zhang G S. Graphene oxide in the water environment could affect tetracycline-antibiotic resistance[J]. Chemosphere, 2017, 183:197-203 Zou W, Li X K, Lai Z Y, et al. Graphene oxide inhibits antibiotic uptake and antibiotic resistance gene propagation[J]. ACS Applied Materials & Interfaces, 2016, 8(48):33165-33174 Yu W C, Zhan S H, Shen Z Q, et al. Efficient removal mechanism for antibiotic resistance genes from aquatic environments by graphene oxide nanosheet[J]. Chemical Engineering Journal, 2017, 313:836-846 Dickschat J S. Quorum sensing and bacterial biofilms[J]. Natural Product Reports, 2010, 27(3):343-369 Ouyang K, Mortimer M, Holden P A, et al. Towards a better understanding of Pseudomonas putida biofilm formation in the presence of ZnO nanoparticles (NPs):Role of NP concentration[J]. Environment International, 2020, 137:105485 Yang Y, Alvarez P J J. Sublethal concentrations of silver nanoparticles stimulate biofilm development[J]. Environmental Science & Technology Letters, 2015, 2(8):221-226 Xiao X, Zhu W W, Liu Q Y, et al. Impairment of biofilm formation by TiO2 photocatalysis through quorum quenching[J]. Environmental Science & Technology, 2016, 50(21):11895-11902 Chen Y, Zhang G M, Wang H J. Enhancement of photosynthetic bacteria biomass production and wastewater treatment efficiency by zero-valent iron nanoparticles[J]. Journal of Bioscience and Bioengineering, 2020, 130(3):306-310 Wang C, Liu S Q, Hou J, et al. Effects of silver nanoparticles on coupled nitrification-denitrification in suspended sediments[J]. Journal of Hazardous Materials, 2020, 389:122130 Li Z W, Wang X J, Ma B R, et al. Long-term impacts of titanium dioxide nanoparticles (TiO2 NPs) on performance and microbial community of activated sludge[J]. Bioresource Technology, 2017, 238:361-368 Peng M W, Yu X L, Guan Y, et al. Underlying promotion mechanism of high concentration of silver nanoparticles on anammox process[J]. ACS Nano, 2019, 13(12):14500-14510 Amen T W M, Eljamal O, Khalil A M E, et al. Wastewater degradation by iron/copper nanoparticles and the microorganism growth rate[J]. Journal of Environmental Sciences, 2018, 74:19-31 Vaghari H, Jafarizadeh-Malmiri H, Mohammadlou M, et al. Application of magnetic nanoparticles in smart enzyme immobilization[J]. Biotechnology Letters, 2016, 38(2):223-233 Zhang Y F, Shen J Q. Enhancement effect of gold nanoparticles on biohydrogen production from artificial wastewater[J]. International Journal of Hydrogen Energy, 2007, 32(1):17-23 Beckers L, Hiligsmann S, Lambert S D, et al. Improving effect of metal and oxide nanoparticles encapsulated in porous silica on fermentative biohydrogen production by Clostridium butyricum[J]. Bioresource Technology, 2013, 133:109-117 Engliman N S, Abdul P M, Wu S Y, et al. Influence of iron (Ⅱ) oxide nanoparticle on biohydrogen production in thermophilic mixed fermentation[J]. International Journal of Hydrogen Energy, 2017, 42(45):27482-27493 Mohanraj S, Kodhaiyolii S, Rengasamy M, et al. Phytosynthesized iron oxide nanoparticles and ferrous iron on fermentative hydrogen production using Enterobacter cloacae:Evaluation and comparison of the effects[J]. International Journal of Hydrogen Energy, 2014, 39(23):11920-11929 Cervantes-Avilés P, Ida J, Toda T, et al. Effects and fate of TiO2 nanoparticles in the anaerobic treatment of wastewater and waste sludge[J]. Journal of Environmental Management, 2018, 222:227-233 Aguilar-Moreno G S, Navarro-Cerón E, Velázquez-Hernández A, et al. Enhancing methane yield of chicken litter in anaerobic digestion using magnetite nanoparticles[J]. Renewable Energy, 2020, 147:204-213 Ambuchi J J, Zhang Z H, Shan L L, et al. Response of anaerobic granular sludge to iron oxide nanoparticles and multi-wall carbon nanotubes during beet sugar industrial wastewater treatment[J]. Water Research, 2017, 117:87-94 Yang C H, Aslan H, Zhang P, et al. Carbon dots-fed Shewanella oneidensis MR-1 for bioelectricity enhancement[J]. Nature Communications, 2020, 11(1):1379 Kim C, Kim J R,Heo J. Enhancement of bioelectricity generation by a microbial fuel cell using Ti nanoparticle-modified carbon electrode[J]. Journal of Chemical Technology & Biotechnology, 2019, 94(5):1622-1627 -
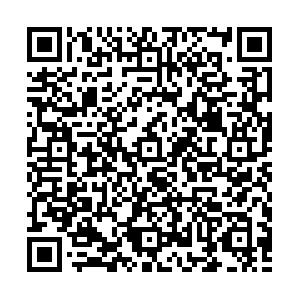
计量
- 文章访问数: 4522
- HTML全文浏览数: 4522
- PDF下载数: 118
- 施引文献: 0